For students
For students
In our working group, we combine extremely different methods, which are already sophisticated in themselves, in order to come a step closer to our goal of being able to record videos on the nanoscale:
Laser
We build and improve our own laser systems to generate exactly the laser pulses we need for our experiments. Unlike traditional amplifier systems, our lasers operate at a repetition rate of 200 kHz, which enables rapid data acquisition in subsequent experiments. The light pulses we generate are typically only a few oscillations of the electric field and thus a few femtoseconds short. A property that makes it easier to control charge carriers directly with the oscillating electric field. We use these laser pulses to illuminate our samples and excite charge carrier dynamics in them.
Vacuum and high-order harmonics
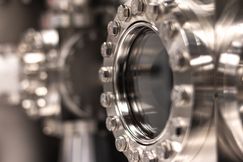
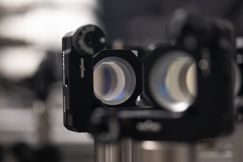
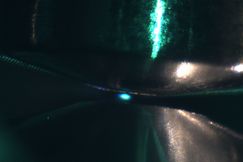
For time-resolved experiments, however, it is not enough to excite dynamics. We also have to interrogate them and use even shorter light pulses for this. In this way, we can directly follow field oscillations of light fields, very similar to an oscilloscope, only now with a bandwidth in the PHz range.
Since it is hardly possible to generate shorter pulses with visible wavelengths, a shorter wavelength or a higher frequency is required. For some years now, the Extreme Ultraviolet (EUV) spectral range has been readily available, very similar to the colors used in the fabrication of semiconductor structures. However, our light pulses in this spectral range have the special property that they are only a few 100 attoseconds short. This makes them among the shortest light pulses ever generated.

By focusing our femtosecond laser pulses into a noble gas, we release individual electrons from the noble gas, accelerate them in the light field and let them be absorbed again by the same noble gas ion. The energy absorbed in the meantime from the optical field is emitted in the form of a high-energy photon. This happens multiple times in each half cycle of the laser field, creating a pulse train of short flashes with high photon energy. This process is called the generation of high-order harmonics (of the laser frequency). To avoid absorption of the light, our experiments take place under ultra-high vacuum conditions where cleanliness plays an important role.
Photoemission electron microscopy
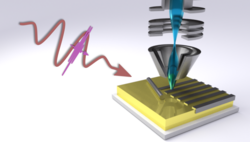
Short laser pulses in the attosecond range make it possible to conduct experiments with extremely high time resolution. What often falls by the wayside is spatial resolution. In certain cases, for example in periodic structures, this is less of a problem. Theoretical modelling or diffractive methods are sufficient to assign the dynamics to individual locations in a sample. In general, however, structures are heterogeneous and it is here that the really exciting processes happen: Charge carriers, driven by a light field, move through a nanostructure, out of it, or from one material region across an interface into another. At these points, charge carriers develop their macroscopic function, but are too fast for previous methods to image them directly.
For this reason, we combine the high time resolution of laser pulses with the high spatial resolution of photoemission electron microscopy. Here, electrons emitted by laser pulses from a sample are examined with regard to their emission location and kinetic energy. An electron microscope is used in which the sample itself forms the electron source. Images of the sample are produced with a resolution of a few 10 nm, in which the movement of charge carriers can be followed with high temporal and energetic resolution. In this way, the two previously separate worlds of resolution, spatial and temporal, are elegantly combined.
Samples
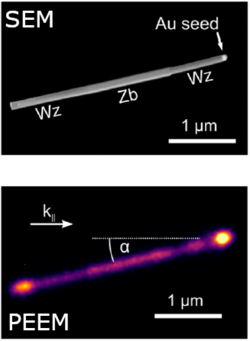
For example, we are investigating how excited electrons and holes in a semiconductor nanowire behave in a spatially and temporally resolved manner. These nanowires are interesting for applications in which the optically excited charge carriers are used, for example, to generate electricity. These "hot electrons", excited beyond the conduction band minimum, are particularly relevant because the excess energy is usually converted into heat and thus lost for electricity generation. Accordingly, a more precise understanding of their behavior may open up the possibility of more efficient use of the solar spectrum.
Wittenbecher, L. et al. Unravelling the Ultrafast Hot Electron Dynamics in Semiconductor Nanowires. ACS Nano 15, 1133-1144 (2021).